FROM:
J Manipulative Physiol Ther 2017 (Jul); 40 (6): 371380 ~ FULL TEXT
William R Reed, DC, PhD, Joel G Pickar, DC, PhD, Randall S Sozio, BS, LATG, Michael A.K. Liebschner, PhD, Joshua W. Little, DC, PhD, and Maruti R Gudavalli, PhD
Palmer Center for Chiropractic Research,
Palmer College of Chiropractic,
Davenport, IA.
Thanks to JMPT for permission to reproduce this Open Access article!
|
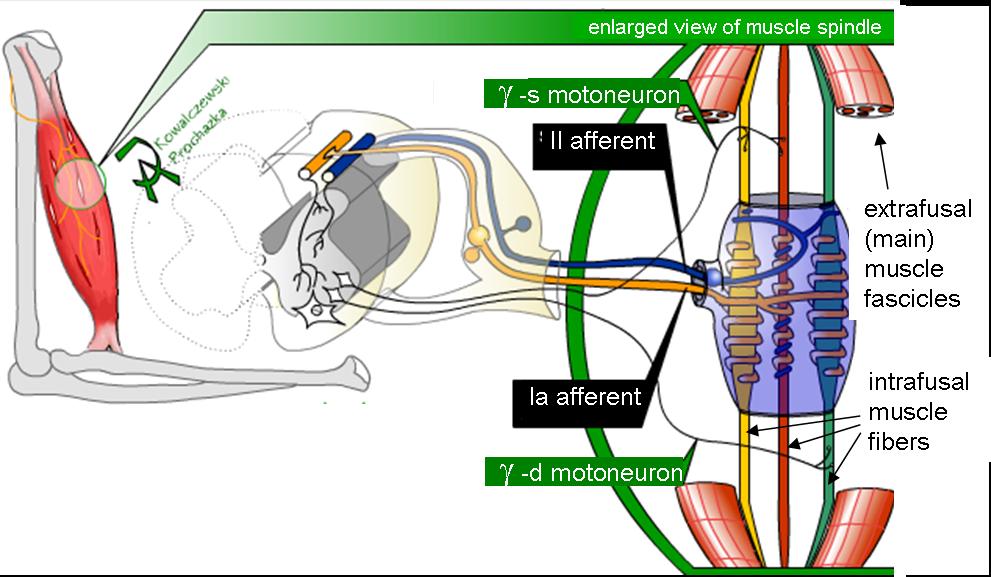
FROM: Wiki
Objectives: The purpose of this preliminary study is to determine muscle spindle response characteristics related to the use of 2 solenoid powered clinical mechanically assisted manipulation (MAM) devices.
Methods: L6 muscle spindle afferents with receptive fields in paraspinal muscles were isolated in 6 cats. Neural recordings were made during L7 MAM thrusts using the Activator V (Activator Methods Int. Ltd., Phoenix, AZ) and/or Pulstar (Sense Technology Inc., Pittsburgh, PA) devices at their 3 lowest force settings. Mechanically assisted manipulation response measures included (a) the time required post-thrust until the first action potential, (b) differences in mean frequency (MF) and mean instantaneous frequency (MIF) 2 seconds before and after MAM, and (c) the time required for muscle spindle discharge (MF and MIF) to return to 95% of baseline after MAM.
Results: Depending on device setting, between 44% to 80% (Pulstar) and 11% to 63% (Activator V) of spindle afferents required >6 seconds to return to within 95% of baseline MF values; whereas 66% to 89% (Pulstar) and 75% to 100% (Activator V) of spindle responses returned to within 95% of baseline MIF in <6 seconds after MAM. Nonparametric comparisons between the 22 N and 44 N settings of the Pulstar yielded significant differences for the time required to return to baseline MF and MIF.
Conclusion: Short duration (<10 ms) MAM thrusts decrease muscle spindle discharge with a majority of afferents requiring prolonged periods (>6 seconds) to return to baseline MF activity. Physiological consequences and clinical relevance of described MAM mechanoreceptor responses will require additional investigation.
Keywords: Afferent Neurons; Muscle Spindles; Spinal Manipulation; Spine.
From the FULL TEXT Article:
Introduction
Low back pain (LBP) continues to be a major societal health problem and prevalence rates are only expected to increase with the aging of the American population. [1, 2] More than 80% of the population will experience an episode of LBP during their lives, with 20 to 40% experiencing a reoccurrence of a LBP episode within 1 year after the initial onset in working populations. [3, 4] LBP can be treated with multiple approaches including non-pharmacological, pharmacological, and surgical interventions. The efficacy, favorable side-effect profile, cost-effectiveness and high patient satisfaction associated with the non-pharmacological approach of spinal manipulation have led to multiple clinical practice guidelines and evidence reports recommending spinal manipulation for certain types of acute and chronic LBP. [511] However, the appropriate use and clinical efficacy of spinal manipulation could be greatly enhanced if underlying peripheral and central neurophysiological mechanisms were clearly established. Accomplishing these objectives will require combined contributions from both basic and clinical investigations.
Spinal manipulation traditionally involves applying a single, short lever, high velocity low amplitude (HVLA) thrust of short duration to a targeted dysfunctional vertebral motion segment and/or joint. HVLA thrusts can be delivered manually with direct hand contact (?150ms thrust duration) or using commercially available mechanical-assisted devices (?10ms thrust duration). [1218] Peak forces achieved during a manipulative thrust can vary dramatically depending upon anatomical location of treatment, localized tissue compliance, clinician and/or patients physical traits, spinal manipulative technique, mechanical-assisted device used and/or device setting. [1525] A distinct advantage mechanically-assisted manipulation (MAM) offers over manual spinal manipulation is that thrust velocity and thrust magnitude can be standardized. MAM is thought to be an effective alternative to manual thrust manipulation based upon both comparable measures of vertebrae movements [12, 2630] and similar clinical outcomes reported in a few smaller studies. [3134] A recent randomized clinical trial comparing manually-delivered spinal manipulation and MAM indicated differences in short-term clinical efficacy, [35] thereby suggesting that distinct physiological mechanisms of action might be responsible for the underlying therapeutic effects of these two types of HVLA spinal manipulation.
Proposed physiological mechanisms of action by which spinal manipulation of any type ameliorates musculoskeletal pain include stretch reflex inhibition generated by stretch-induced stimulation of mechanoreceptors or nociceptors in the facet joint capsule and/or the surrounding spinal tissues (muscles, ligaments, discs). [3643] In humans, the Hoffmann reflex (H-reflex) and stretch reflexes have been used to provide a neurophysiological index of ?-motoneuron excitability. A commonality shared by a majority of spinal manipulative studies that investigated H-reflex and stretch reflex responses is the concept that manual therapies mechanistically act by reflexively reducing central ?-motoneuron activity via modulating the sensitivity of muscle spindle afferents to muscle stretch. [37, 4148] Using a computer-controlled feedback motor, we have previously investigated the relationship between various biomechanical parameters of spinal manipulation (thrust duration, thrust magnitude, thrust rate, thrust contact site etc.) and paraspinal muscle spindle responsiveness in both laminectomy-only and acute facet joint fixation animal models. [4955] Time to peak impulse in the majority of these animal studies ranged from 75150ms which is more closely aligned with manually-delivered spinal manipulation compared to the faster time to peak impulse of 27ms reported among MAM devices. [15, 18] This extremely short time to peak impulse with MAM devices may result in unique muscle spindle responses and/or central reflex-related mechanisms as these particular mechanoreceptors are sensitive to the rate of muscle stretch.
Figure 1
|
To begin to address the neurophysiological impact of these extremely short MAM thrust durations, we recently conducted a pilot study in a single feline preparation to determine the feasibility of recording muscle spindle afferent responses using an instrumented spring-loaded hammer MAM device (Activator IV ; Activator Methods Int. Ltd., Phoenix, AZ). [56] Despite recording from a limited number of muscle spindle afferents, this pilot study clearly demonstrated that a diverse set of muscle spindle afferent responses are elicited using clinical MAM devices. Post-thrust MAM neural responses ranged from a slight diminution of muscle afferent discharge compared to pre-thrust, to a complete cessation of afferent discharge for nearly 3 seconds. [56] The purpose of this preliminary study is to begin to characterize the temporal effects of two electromagnetic solenoid-powered MAM devices (Activator Methods Int. Ltd., Phoenix, AZ [Figure 1A] and Pulstar; Sense Technology Inc., Pittsburgh, PA [Figure 1B]) on muscle spindle discharge to inform future studies.
Methods
General Surgery
All experiments were reviewed and approved by the Palmer Institutional Animal Care and Use Committee #20120601. Electrophysiological activity in 6 muscle spindle afferent fibers was obtained during MAM delivered to 6 deeply anesthetized male cats weighing between 4.4 and 6.0kg [mean 5.3 (SD 0.6)]. Data collected using these electromagnetic MAM devices were from experimental preparations associated with a separate larger study investigating the relationship between intervertebral fixation and spinal manipulation as delivered by a computer controlled feedback motor. [55] The surgical and experimental procedures, including monitoring the preparations anesthetic level and physiological status as well as methods of isolating paraspinal muscle spindle afferents have been previously described in detail elsewhere [51, 52, 55, 57, 58] and thus are only presented here briefly. Anesthesia was induced with isoflurane and maintained with sodium pentobarbital (35mg/kg, iv). Catheters were placed in the common carotid artery and external jugular vein to monitor blood pressure, introduce fluids and anesthesia. The trachea was intubated, and the cat ventilated mechanically. Arterial gases and body temperature were monitored and maintained within the reference range (pH 7.327.43; PCO2, 3237mmHg; PO2, >85mmHg, 3839°C). Body temperature was continuously monitored and maintained between 3839°C using a water-heating pad/radiant heat. Additional Nembutal (5 mg/kg) was administered (iv) upon withdrawal reflex to noxious pinching of the toe pad or when mean arterial pressure increased above 100mmHg.
Figure 2
|
To better isolate trunk muscle afferents, the right sciatic nerve was cut to reduce afferent input from the hindlimb. The lumbar spine was mechanically secured at the L4 spinous and the iliac crests. A midline skin incision was made and an L5 laminectomy was performed to expose the L6 dorsal rootlets while leaving all intervertebral joints intact. The L6 dorsal rootlets were cut close to the spinal cord and thin filaments were teased using forceps until impulse activity from a single trunk muscle spindle afferent was identified (Figure 2). Action potentials were recorded using a PC-based data acquisition system (Spike 2; Cambridge Electronic Design, Cambridge, UK). Afferents were identified as muscle spindles based on their increased discharge to succinylcholine (100200mg/kg intra-arterially; Butler Schein, Dublin, OH), decreased discharge to electrically induced muscle contraction, and/or sustained response to a fast vibratory stimulus. At the end of the experiment, animals were euthanized by an intravenous overdose of pentobarbital.
Mechanically-Assisted Manipulation
Both the Activator V and Pulstar are commercially available hand-held clinical MAM devices. The Activator V has four progressive thrust magnitude settings and requires that the device tip to completely retracted during pre-load (~2.9N) before a single mechanical impulse can delivered at any device setting. When tested against a stiff spinal tissue analog (258.07 N/mm), Activator V delivered mean peak forces of 52, 68, 112, and 155N at settings 14 respectively in hand-held operations. [18] Device testing on more compliant spinal tissue analog (30.22 N/mm) resulted in mean peak forces of 35, 63, 102N at settings 13 respectively. [18] The Pulstar device has 7 settings and can be used to deliver either single or multiple high velocity mechanical impulses (22155N) once the pre-defined preload value of ~15N has been successfully applied. [13, 14] The three lowest settings on the Pulstar device deliver approximate peak forces of 22, 44, and 67N respectively. In the present study, only single impulses were delivered using the Pulstar device. All MAM thrusts were delivered perpendicularly onto the exposed fascia overlying the cats L7 spinous process as previously described. [56] The L7 spinous was chosen to receive MAM due to the potential increased risk for repetitive L6 manipulations to tear the L6 afferent fiber off the recording electrode. Typically, three HVLA thrusts were delivered per afferent at each of the three lowest settings of the respective MAM devices (18 thrusts total) with 5 minute intervals between thrusts. Use of MAM devices and device settings (thrust magnitudes) were randomized to minimize any possible ordering effects. At times, not all MAM test protocols were completed before the neural recording was lost. Four afferents received L7 HVLA thrusts from both MAM devices while two afferents received L7 HVLA thrusts from only the Pulstar device.
Data Analysis
Table 1
Table 4
|
Muscle spindle discharge was quantified as mean frequency (MF) and mean instantaneous frequency (MIF). MIF was calculated by taking the reciprocal of the time interval between successive action potentials. Baseline MF/MIF activity measures were obtained during a 2 second period that immediately preceded MAM device contact and/or soft tissue preload as in previous studies [5153, 55] (Figure 2). The following three response measures were determined: 1) the time required post-thrust until the 1st action potential, 2) MF and MIF differences between 2s post-MAM thrust and 2s of baseline activity, and 3) the time required for spindle discharge to return to 95% of baseline MF/MIF for a minimum period of 1s following MAM (Fig. 2). The descriptive data presented in Tables 14 are mean responses or time durations from 8 to 18 individual MAM thrusts delivered at a given device setting. Statistical comparisons were made between device settings of a given MAM device using the mean afferent responses to the MAM thrusts delivered at each device setting. For the Pulstar device, nonparametric comparisons of magnitude settings were performed on ranked data to minimize the effect of increased variability coupled with smaller sample size using the Wilcoxon Signed Ranks Test. Statistical comparisons for the Activator V device were not performed due to fewer afferents tested with this device. Statistical significance was set at P < 0.05 and data variance is shown as standard error of the mean (SEM) unless otherwise noted.
Results
Responses to MAM from 6 muscle spindle afferents with receptive fields located in paraspinal muscles (longissimus or multifidus) were recorded in 6 cats. Mean muscle spindle response characteristics for individual MAM thrust settings are presented in Tables 14. Note that applied forces, pulse widths, device preload settings, etc. are not equivalent between devices thus direct comparisons between MAM devices are inappropriate.
Activator V Device
An example of a raw recording from a single afferent to MAM using the Activator at the 2nd device setting is shown in Figure 2. Progressive increases in Activator V MAM thrust forces typically resulted in longer mean delays until the 1st post-thrust action potential (Table 1). Compared to the 2s of baseline activity, MAM resulted in mean decreases in spindle discharge for both MF and MIF during the 2s post-MAM thrust. Progressive increases in MAM thrust forces did not necessarily result in greater changes in MF/MIF between 2s after MAM and 2s baseline measures. The greatest mean change in MF and MIF occurred at the 2nd device setting on the Activator V (Table 1). Regarding the time required for MF/MIF to return to 95% of baseline following Activator MAM (Table 2), 2545% of the afferents tested returned to baseline MF within 2s post-MAM and no afferent required longer than 8s to return to baseline MF at device setting 1. However at ActivatorV device settings 2 and 3, over 33% of afferents required >10s thus creating a distinct separation of those afferents requiring shorter (<2s) or longer (>10s) durations to return to baseline MF. Regardless of device setting, the vast majority of afferents (6789%) tested with the Activator V returned to baseline MIF within 2s and no afferent required a period greater than 8s (Table 2).
Pulstar Device
An example of 3 raw recordings from a single muscle spindle afferent to MAM using the Pulstar device at settings of 22, 44, and 67N are shown in Figure 3. The time required until the first action potential was similar at 44 and 67N. Compared to the 2s of baseline activity, Pulstar MAM resulted in mean decreases in spindle discharge for both MF and MIF during the 2s post-MAM thrust at all thrust magnitudes (Table 3). Regarding the time required for MF/MIF to return to 95% of baseline following MAM (Table 4), it is interesting to note that over 33% of afferents tested required more than 10s to return to baseline MF at all Pulstar device settings tested. The delay in return to baseline MIF with the Pulstar was broadly distributed across post- MAM time intervals at each of the three device settings tested (Table 4). Nonparametric comparisons between outcomes among the different Pulstar device settings yielded significant differences between 22 and 44N settings for the time required to return to 95% of baseline MF and MIF (P < .05). The mean time required to return to baseline MF was 8.4s (1.9) and 14.8s (2.6) for the 22 and 44N setting respectively. The mean time required to return to baseline MIF was 3.4s (0.3) and 5.2s (0.8) for the 22 and 44N Pulstar device settings.
Discussion
Basic investigations related to neurophysiological changes associated with MAM are important in order to establish underlying mechanisms responsible for spinal manipulation efficacy and to optimize treatment regimens for the 4060% of chiropractic and/or other manual therapy clinicians in the United States, Canada, Britain, Belgium, New Zealand and Australia who use these mechanical manipulation devices in some capacity of patient care. [5964] Despite recent strides being made in determining both the mechanical characteristics and/or physiological effects of MAM, [13, 15, 17, 18, 25, 56, 6574 ] to our knowledge, this study is the first to record muscle spindle response evoked using solenoid powered hand-held clinical MAM device technology and only the second to investigate muscle spindle response using any type of MAM device.
This study confirms findings of a previous report using a spring-loaded MAM device (Activator IV) demonstrating that a range of muscle spindle responses are elicited by MAM. [56] The combined tissue preload and extremely short MAM thrust durations elicited high frequency discharge from paraspinal muscle spindle afferents followed by a decrease in post-MAM activity. The progressive increase in applied forces typically increased the time required until the 1st action potential (Tables 1, 3). However, this relationship between applied force and delayed neural response did not necessarily prove to be the case with regard to the amount of time required to return to 95% of baseline MF or MIF. Nonparametric analyses of afferent response to Pulstar thrusts indicated significant differences between 22 and 44N thrust settings with greater time being required to MF/MIF to return to 95% baseline levels for the 44N MAM thrust but not between the 44 and 67N thrust settings. There appeared to be distinct subpopulations of afferent responses with some returning to baseline activity levels relatively rapidly (<4s) while others required much longer periods (>10s) (Tables 2, 4). It is not known whether these differences are due to inherit differences related to muscle spindle intrafusal fibers types such as bag1, bag2 and/or chain fibers (see Reed et al. [75] for greater detail) or to other biological factors.
In the present study we provide post-MAM data regarding changes in both MF and MIF (Tables 14), while in the past [51, 54] we have primarily focused our attention on MIF changes during the thrust phase (time to peak force) of the spinal manipulation. Due to the lack of tissue preload standardization and hand-held vs computer controlled feedback motor clinical delivery methods, we have chosen to focus on the post-thrust MAM muscle spindle response instead of the spindle response elicited during the thrust. Since muscle spindle afferents typically discharge tonically while at rest, we thought providing the time required to return to 95% baseline activity for both MF and MIF might provide a more complete picture of post-thrust MAM response. As clearly illustrated in Fig. 2, MF and MIF recovery do not always occur within close proximity, but generally speaking, these two response measures typically occurred within 0.52s of each other. Comparisons for the Pulstar device indicated significant increases for both MF and MIF when comparing time to return to baseline spindle activity following MAM thrusts delivered at the 22 and 44N device settings. We are uncertain as to how this prolonged delay in return to baseline discharge following MAM affects central interneuron, gamma and/or alpha-motoneuron activity and whether or not these delay differences contribute to the physiological mechanisms or clinical efficacy of spinal manipulation. Answers to these questions will require additional basic and clinical investigation using MAM devices.
Limitations
Preload forces and preload durations were not standardized and MAM devices were not rigidly secured or instrumented to record the actual forces delivered. Instead, MAM devices were hand-held as typically used in clinical settings and care was taken to apply the minimum preload necessary to trigger the respective MAM device. While we tested the 3 lowest settings on each clinical device (with peak forces as low as 22N), peak forces were not scaled to feline bodyweight per se. While aspects of a scaling requirement have validity, determining the best scaling parameters is far from clear. Localized thrust forces are quickly dampened by surrounding viscoelastic in vivo tissues and biological cell response does not scale with body weight. [18, 76] Compared to direct load cell MAM testing, recent evidence using spinal tissue analogs demonstrate that applied MAM peak forces are substantially lower (up to 59%) based on localized viscoelastic tissue compliance combined with additional compliance from the hand/wrist/forearm when devices are hand-held. [18] It should be noted that L7 MAM thrust procedures were not intense enough to tear nearby L6 finely teased afferent fibers from the recording electrode and no tissue damage or spinal fractures occurred with multiple MAM thrusts. While future studies should consider scaling of applied MAM peak forces, this study highlights the need to determine the actual forces being transmitted through the spine and surrounding tissues in vivo and suggests that these applied forces are likely substantially lower than those reported in the literature following testing on highly idealized beam structures. [18]
Conclusions
This study marks the first time that paraspinal muscle spindle response characteristics related to the delivery of extremely short duration (<10ms) solenoid powered MAM thrusts has been reported. Overall, MAM thrusts resulted decreased muscle spindle discharge with a majority of afferents requiring prolonged periods of time (>6s) to return to pre-thrust levels of MF/MIF activity. This neurophysiological effect of MAM far outlasts the 27ms stimulus duration, but determination as to how this prolonged delay in return to baseline activity levels impacts spinal or supraspinal mechanosensory processing or clinical outcomes will require additional investigation.
Key Findings
This study found that short duration (<10ms) mechanically-assisted lumbar manipulative thrusts decreases muscle spindle discharge relative to baseline activity,
It required prolonged periods (>6s) for paraspinal muscle spindles to return to their pre-thrust resting discharge activity levels following short duration (<10ms) mechanically-assisted spinal manipulative thrusts.
This study suggests that subpopulations of muscle spindle afferents respond differently to short duration mechanically-assisted spinal manipulative thrusts.
These findings may become important to patient care if future studies show that mechanosensory response is a mechanism that contributes to the clinical efficacy of spinal manipulation.
|
Acknowledgments
The authors would like to thank Darlene Burke for statistical analyses support. The authors would also like to thank Arlan Fuhr of Activator Methods International and Christian Evans of Sense Technology Inc. for providing the MAM devices used in this study.
FUNDING SOURCES
This work was supported by the NIH National Center for Complementary and Integrative Health (K01AT005935) to WRR and was conducted in a facility with support from the NIH National Center for Research Resources under Research Facilities Improvement Grant Number C06RR15433.
Contributorship
Concept development (provided idea for the research) WRR, JGP, MRG
Design (planned the methods to generate the results) WRR,
Supervision (provided oversight, responsible for organization and implementation, writing of the manuscript) WRR
Data collection/processing (responsible for experiments, patient management, organization, or reporting data) WRR, RSS
Analysis/interpretation (responsible for statistical analysis, evaluation, and presentation of the results) WRR, JWL, JGP, MRG, MAL
Literature search (performed the literature search) WRR, MAL
Writing (responsible for writing a substantive part of the manuscript) WRR
Critical review (revised manuscript for intellectual content, this does not relate to spelling and grammar checking) WRR, JWL, RSS, JGP, MRG, MAL
CONFLICTS OF INTEREST
No conflicts of interest were reported for this study.
References:
Freburger JK, Holmes GM, Agans RP, et al.
The rising prevalence of chronic low back pain.
Arch Intern Med. 2009;169:2518
Guo HR, Tanaka S, Halperin WE, et al.
Back pain prevalence in US industry and estimates of lost workdays.
Am J Public Health. 1999;89:102935
Rubin DI.
Epidemiology and risk factors for spine pain.
Neurologic clinics. 2007;25:35371
van Tulder M, Koes B, Bombardier C.
Low back pain.
Best Pract Res Clin Rheumatol. 2002;16:76175
Childs JD, Cleland JA, Elliott JM, et al.
Neck pain: Clinical practice guidelines linked to the International
Classification of Functioning, Disability, and Health from the Orthopedic
Section of the American Physical Therapy Association.
J Orthop Sports Phys Ther. 2008;38:A1a34
Pillastrini P, Gardenghi I, Bonetti F, et al.
An Updated Overview of Clinical Guidelines for
Chronic Low Back Pain Management in Primary Care
Joint Bone Spine 2012 (Mar); 79 (2): 176185
Bronfort G, Haas M, Evans R, Leiniger B, Triano J.
Effectiveness of Manual Therapies: The UK Evidence Report
Chiropractic & Osteopathy 2010 (Feb 25); 18 (1): 3
Chou R, Qaseem A, Snow V, Casey D, Cross JT Jr., Shekelle P, Owens DK:
Diagnosis and Treatment of Low Back Pain: A Joint Clinical Practice Guideline
from the American College of Physicians and the American Pain Society
Annals of Internal Medicine 2007 (Oct 2); 147 (7): 478491
Chou R, Huffman LH; American Pain Society.
Nonpharmacologic Therapies for Acute and Chronic Low Back Pain:
A Review of the Evidence for an American Pain Society/
American College of Physicians Clinical Practice Guideline
Annals of Internal Medicine 2007 (Oct 2); 147 (7): 492504
Chou R, Atlas SJ, Stanos SP, Rosenquist RW.
Nonsurgical Interventional Therapies for Low Back Pain:
A Review of the Evidence for an American Pain Society
Clinical Practice Guideline
Spine (Phila Pa 1976). 2009 (May 1); 34 (10): 10781093
Dagenais S, Tricco AC, Haldeman S.
Synthesis of Recommendations for the Assessment and Management
of Low Back Pain From Recent Clinical Practice Guidelines
Spine J. 2010 (Jun); 10 (6): 514529
Keller ST, Colloca CJ, Moore RJ, Gunzburg R, Harrison DE, Harrison DD.
Three-dimensional Vertebral Motions Produced by
Mechanical Force Spinal Manipulation
J Manipulative Physiol Ther. 2006 (Jul); 29 (6): 425436
Evans JM.
Differential compliance measured by the function recording and
analysis system in the assessment of vertebral subluxation.
J Vertebral Subluxation Res. 1998;2:1521
Leach RA, Parker PL, Veal PS:
PulStar Differential Compliance Spinal Instrument: A Randomized
Interexaminer and Intraexaminer Reliability Study
J Manipulative Physiol Ther 2003 (Oct); 26 (8): 493501
Colloca CJ, Keller TS, Black P, Normand MC, Harrison DE, Harrison DD:
Comparison of Mechanical Force of Manually Assisted
Chiropractic Adjusting Instruments
J Manipulative Physiol Ther 2005 (Jul); 28 (6): 414422
Herzog W:
The Biomechanics of Spinal Manipulation
J Bodyw Mov Ther. 2010 (Jul); 14 (3): 280286
Kawchuk GN, Herzog W.
Biomechanical characterization (fingerprinting) of five
novel methods of cervical spine manipulation.
J Manipulative Physiol Ther. 1993;16:5737
Liebschner MA, Chun K, Kim N, et al.
In Vitro Biomechanical Evaluation of Single Impulse and Repetitive Mechanical Shockwave Devices Utilized for
Spinal Manipulative Therapy
Annals of Biomedical Engineering 2014 (Dec); 42 (12): 25242536
Herzog W, Conway PJ, Kawchuk GN, et al.
Forces exerted during spinal manipulative therapy.
Spine. 1993;18:120612
Hessell BW, Herzog W, Conway PJ, et al.
Experimental measurement of the force exerted during
spinal manipulation using the Thompson technique.
J Manipulative Physiol Ther. 1990;13:44853
Cambridge ED, Triano JJ, Ross JK, et al.
Comparison of force development strategies of
spinal manipulation used for thoracic pain.
Man Ther. 2012;17:2415
Forand D, Drover J, Suleman Z, et al.
The forces applied by female and male chiropractors during thoracic spinal manipulation.
J Manipulative Physiol Ther. 2004;27:4956
Triano J, Schultz AB.
Loads transmitted during lumbosacral spinal manipulative therapy.
Spine. 1997;22:195564
Triano JJ.
Biomechanics of Spinal Manipulative Therapy
Spine J. 2001 (Mar); 1 (2): 121130
Kawchuk GN, Prasad NG, McLeod RC, et al.
Variability of force magnitude and force duration in manual
and instrument-based manipulation techniques.
J Manipulative Physiol Ther. 2006;29:6118
Nathan M, Keller TS.
Measurement and analysis of the in vivo posteroanterior impulse response
of the human thoracolumbar spine: a feasibility study.
J Manipulative Physiol Ther. 1994;17:43141
Cramer GD, Gregerson DM, Knudsen JT, et al.
The effects of side-posture positioning and spinal adjusting on the
lumbar Z joints: a randomized controlled trial with sixty-four subjects.
Spine. 2002;27:245966
Gal J, Herzog W, Kawchuk G, et al.
Movements of vertebrae during manipulative thrusts to unembalmed human cadavers.
J Manipulative Physiol Ther. 1997;20:3040
Keller TS, Colloca CJ, Gunzburg R.
Neuromechanical characterization of in vivo lumbar
spinal manipulation. Part I. Vertebral motion.
J Manipulative Physiol Ther. 2003;26:56778
Maigne JY, Guillon F.
Highlighting of intervertebral movements and variations of intradiskal
pressure during lumbar spine manipulation: a feasibility study.
J Manipulative Physiol Ther. 2000;23:5315
Gemmell HA, Jacobson BH.
The immediate effect of activator vs. meric adjustment on
acute low back pain: a randomized controlled trial.
J Manipulative Physiol Ther. 1995;18:4536
Schneider MJ, Brach J, Irrgang JJ, et al.
Mechanical vs manual manipulation for low back pain:
an observational cohort study.
J Manipulative Physiol Ther. 2010;33:193200
Shearar KA, Colloca CJ, White HL.
A randomized clinical trial of manual versus mechanical force
manipulation in the treatment of sacroiliac joint syndrome.
J Manipulative Physiol Ther. 2005;28:493501
Wood TG, Colloca CJ, Mathews R.
A Pilot Randomized Clinical Trial on the Relative Effect of Instrumental
(MFMA) Versus Manual (HVLA) Manipulation in the Treatment
of Cervical Spine Dysfunction
J Manipulative Physiol Ther 2001 (May); 24 (4): 260271
Schneider, M, Haas, M, Glick, R, Stevans, J, and Landsittel, D.
Comparison of Spinal Manipulation Methods and Usual Medical Care
for Acute and Subacute Low Back Pain:
A Randomized Clinical Trial
Spine (Phila Pa 1976). 2015 (Feb 15); 40 (4): 209217
Bialosky JE, Bishop MD, Price DD, Robinson ME, George SZ.
The Mechanisms of Manual Therapy in the Treatment of
Musculoskeletal Pain: A Comprehensive Model
Man Ther. 2009 (Oct); 14 (5): 531538
Clark B, Goss D, Walkowski S, Hoffman R, Ross A, Thomas J.
Neurophysiologic Effects of Spinal Manipulation in Patients
With Chronic Low Back Pain
BMC Musculoskelet Disord. 2011 (Jul 22); 12: 170
Cramer GD, Ross K, Raju PK, et al.
Quantification of cavitation and gapping of lumbar
zygapophyseal joints during spinal manipulative therapy.
J Manipulative Physiol Ther. 2012;35:61421
Fuhr AW.
The Activator Method. 2.
St. Louis: Mosby Elsevier; 2009
Pickar JG.
Neurophysiological Effects of Spinal Manipulation
Spine J (N American Spine Society) 2002 (Sep); 2 (5): 357371
Korr IM.
The Neurobiologic Mechanisms in Manipulative Therapy
ed. New York: Plenum Press; 1978
Dishman JD, Dougherty PE, Burke JR.
Evaluation of the effect of postural perturbation on motoneuronal
activity following various methods of lumbar spinal manipulation.
Spine J. 2005;5:6509
Dishman JD, Bulbulian R.
Spinal reflex attenuation associated with spinal manipulation.
Spine. 2000;25:251924. discussion 25
Bulbulian R, Burke J, Dishman JD.
Spinal reflex excitability changes after lumbar spine passive flexion mobilization.
J Manipulative Physiol Ther. 2002;25:52632
Suter E, McMorland G, Herzog W.
Short-term effects of spinal manipulation on H-reflex
amplitude in healthy and symptomatic subjects.
J Manipulative Physiol Ther. 2005;28:66772
Niazi IK, Turker KS, Flavel S, Kinget M, Duehr J, Haavik H.
Changes in H-reflex and V-waves Following Spinal Manipulation
Experimental Brain Research 2015 (Apr); 233 (4): 11651173
Dishman JD, Cunningham BM, Burke J.
Comparison of tibial nerve H-reflex excitability after
cervical and lumbar spine manipulation.
J Manipulative Physiol Ther. 2002;25:31825
Dishman JD, Burke J.
Spinal reflex excitability changes after cervical and
lumbar spinal manipulation: a comparative study.
Spine J. 2003;3:20412
Pickar JG, Kang YM.
Paraspinal muscle spindle responses to the duration of a
spinal manipulation under force control.
J Manipulative Physiol Ther. 2006;29:2231
Pickar JG, Sung PS, Kang YM, et al.
Response of lumbar paraspinal muscles spindles is greater to spinal
manipulative loading compared with slower loading under length control.
Spine J. 2007;7:58395
Reed, W. R., Cao, D. Y., Long, C. R., Kawchuk, G. N., & Pickar, J. G.
Relationship between Biomechanical Characteristics of Spinal Manipulation
and Neural Responses in an Animal Model: Effect of Linear Control
of Thrust Displacement versus Force, Thrust Amplitude,
Thrust Duration, and Thrust Rate
Evidence-Based Complementary and Alternative Medicine 2013 (Jan 20); 492039
Reed, W. R., Long, C.R., & Pickar, J. G.
Effects of Unilateral Facet Fixation and Facetectomy on Muscle Spindle
Responsiveness During Simulated Spinal Manipulation
in an Animal Model
J Manipulative Physiol Ther. 2013 (Nov); 36 (9): 585594
Reed WR, Long CR, Kawchuk GN, et al.
Neural responses to the mechanical parameters of a high-velocity,
low-amplitude spinal manipulation: effect of preload parameters.
J Manipulative Physiol Ther. 2014;37:6878
Reed, W. R., Long, C. R., Kawchuk, G. N., & Pickar, J. G.
Neural Responses to the Mechanical Characteristics of High Velocity,
Low Amplitude Spinal Manipulation: Effect of Specific Contact Site
Manual Therapy 2015 (Dec); 20 (6): 797804
Reed, W. R., & Pickar, J. G.
Paraspinal Muscle Spindle Response to Intervertebral Fixation and
Segmental Thrust Level During Spinal Manipulation in an Animal Model
Spine (Phila Pa 1976) 2015 (Jul 1); 40 (13): E752759
Reed W. R., Liebschner M. A., Sozio R. S., Pickar J. G., Gudavalli M. R.
Neural Response During a Mechanically Assisted Spinal
Manipulation in an Animal Model: A Pilot Study
J Nov Physiother Phys Rehabil. 2015 (Sep); 2 (2): 2027
Cao DY, Reed WR, Long CR, et al.
Effects of thrust amplitude and duration of high-velocity, low-amplitude
spinal manipulation on lumbar muscle spindle responses
to vertebral position and movement.
J Manipulative Physiol Ther. 2013;36:6877
Pickar JG.
An in vivo preparation for investigating neural responses to
controlled loading of a lumbar vertebra in the anesthetized cat.
J Neurosci Methods. 1999;89:8796
Examiners NBoC.
Job Analysis of Chiropractic in Canada 1994
a project report, survey analysis and summary of the
practice of chiropractic in Canada.
Greeley, Colorado, USA: 1994.
National Board of Chiropractic Examiners
Examiners NBoC.
Job Analysis of Chiropractic by State 1994
a project report, survey analysis, and summary of the
practice of chiropractic within the United States.
Greeley, Colorado, USA: 1994.
National Board of Chiropractic Examiners
Examiners NBoC.
Job Analysis of Chiropractic 2005
a project report, survey analysis and summary of the
practice of chiropractic in the United States.
Greeley, Colorado, USA: 2005.
National Board of Chiropractic Examiners
Ailliet L, Rubinstein SM, de Vet HC.
Characteristics of chiropractors and their patients in Belgium.
J Manipulative Physiol Ther. 2010;33:61825
Read DT, Wilson FJ, Gemmell HA.
Activator as a therapeutic instrument: Survey of usage and opinions
amongst members of the British Chiropractic Association.
Clin Chiropr. 2006;9:705
Gleberzon BJ.
Chiropractic name techniques in Canada: A continued look at demographic
trends and their impact on issues of jurisprudence.
J Can Chiro Assoc. 2002;46:24156
Colloca CJ, Keller TS, Gunzburg R, et al.
Neurophysiologic response to intraoperative lumbosacral spinal manipulation.
J Manipulative Physiol Ther. 2000;23:44757
Colloca CJ, Keller TS, Gunzburg R.
Neuromechanical Characterization Of In Vivo Lumbar
Spinal Manipulation. Part II. Neurophysiological Response
J Manipulative Physiol Ther. 2003 (Nov); 26 (9): 579591
Colloca CJ, Keller TS.
Stiffness and neuromuscular reflex response of the human spine to
posteroanterior manipulative thrusts in patients with low back pain.
J Manipulative Physiol Ther. 2001;24:489500
Colloca CJ, Keller TS.
Electromyographic reflex responses to mechanical force,
manually assisted spinal manipulative therapy.
Spine. 2001;26:111724
Colloca CJ, Keller TS, Gunzburg R:
Biomechanical and Neurophysiological Responses to Spinal
Manipulation in Patients With Lumbar Radiculopathy
J Manipulative Physiol Ther. 2004 (Jan); 27 (1): 115
Colloca CJ, Keller TS, Harrison DE, et al.
Spinal manipulation force and duration affect
vertebral movement and neuromuscular responses.
Clin Biomechan. 2006;21:25462
Colloca CJ, Keller TS, Moore RJ, et al.
Effects of disc degeneration on neurophysiological responses during
dorsoventral mechanical excitation of the ovine lumbar spine.
J Electromyogr Kinesiol. 2008;18:82937
Colloca CJ, Keller TS, Moore RJ, et al.
Intervertebral disc degeneration reduces vertebral motion responses.
Spine. 2007;32:E54450
Keller TS, Colloca CJ, Fuhr AW.
Validation of the force and frequency characteristics of the activator
adjusting instrument: effectiveness as a mechanical
impedance measurement tool.
J Manipulative Physiol Ther. 1999;22:7586
Symons BP, Herzog W, Leonard T, et al.
Reflex responses associated with activator treatment.
J Manipulative Physiol Ther. 2000;23:1559
Reed WR, Cao DY, Ge W, et al.
Using vertebral movement and intact paraspinal muscles to determine
the distribution of intrafusal fiber innervation of
muscle spindle afferents in the anesthetized cat.
Exp Brain Res. 2013;225:20515
Liebschner MA.
Biomechanical considerations of animal models used
in tissue engineering of bone.
Biomaterials. 2004;25:1697714.
Return to SUBLUXATION
Return to INSTRUMENT ADJUSTING
Since 5-25-2023
|